Superfluid Helium 3 and the search for new physics
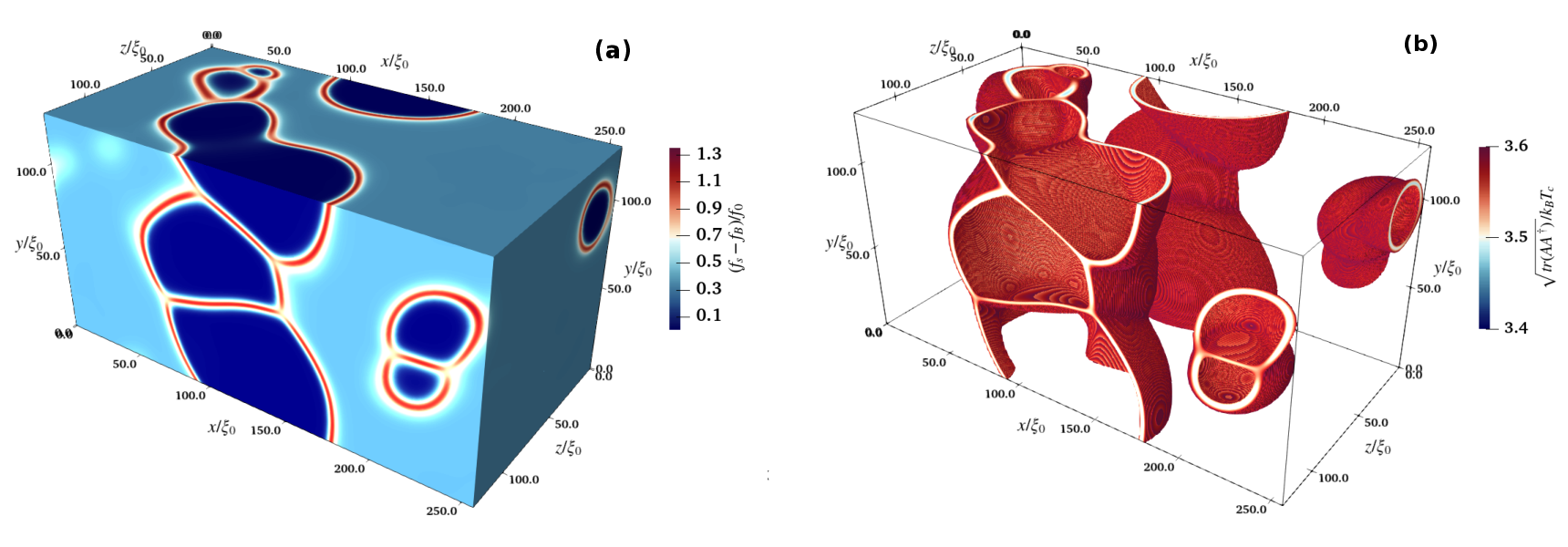
HIP members are working with a UK-based collaboration in a new project to use superfluid helium as laboratory testbed for cosmology. The collaboration, Quantum-Enhanced Superfluid Technology for Dark Matter and Cosmology (QUEST-DMC), exploits the special properties of the superfluid phase of the lighter isotope of helium, 3He, to build more sensitive detectors for dark matter, and to study theories of first order phase transitions, which apply equally to superfluid 3He and the early universe. The collaboration, funded by the Science and Technology Facilities Council, brings together the Universities of Lancaster and Sussex, Royal Holloway, University of London, and experts from all over the world, including early universe cosmologists at HIP and the 3He specialists at the low temperature laboratory in Aalto University.
New physics, gravitational waves and nucleation theory
The Standard Model of particle physics (SM), our best description of nature so far, does not predict a first order transition as the early universe expands and cools. On the other hand, the majority of particle physicists are convinced that the SM is not the ultimate description of matter and interactions: it has no particle which could make up the dark matter, and it does not explain why there is more matter than antimatter in the universe. The problems with the Standard Model motivate extending it. The study of extensions to the SM is known as BSM (beyond the Standard Model) physics. BSM physics predicts new particles and the search for new particles is one of the top priorities of HIP researchers working at the Large Hadron Collider at CERN. It also emerges that many BSM theories predict a first order phase transition in the early universe, and that the transition will lead to the generation of gravitational waves (GWs) [citation]. As a result, the search for gravitational waves from the early universe then becomes a complementary search for BSM physics. HIP researchers are also involved in the search for these gravitational waves at the future space-based observatory LISA, recently adopted by the European Space Agency for launch next decade.
In order to search for gravitational waves, one has to know what one is looking for, as they are very weak signals which can easily be confused with the overlapping waves from closer astrophysical sources. Different sources have characteristic frequency spectra, and so there is intensive work at HIP and around the world on improving the accuracy of the gravitational wave predictions. The first thing one needs to know about an early universe transition is how fast it happened, as this sets the dominant frequency of the signal. The study of the transition rate is known as nucleation theory, as the transition happens by the nucleation of bubbles of a new phase of matter, in a similar way to how bubbles of carbon dioxide appear in a soft drink as the cap is unscrewed and the pressure reduced. It turns out that nucleation theory is essentially the same in a BSM theory as it is in superfluid 3He: therefore one can test the theory against experiments in laboratory systems.
The A-B nucleation puzzle and the QUEST-DMC project
In the absence of magnetic field, superfluid 3He has two phases and a first order transition between them as shown in Figure 1. Historically, these two phases are called A phase and B phase. In zero magnetic field, the A phase is stable only at high pressure, but as the magnetic field is increased, the A phase becomes more stable and for fields above 0.6 tesla, it is the stable superfluid phase at any temperature and pressure. A phase is also the stable phase in a thin film trapped between two smooth walls, a technique known as confinement.
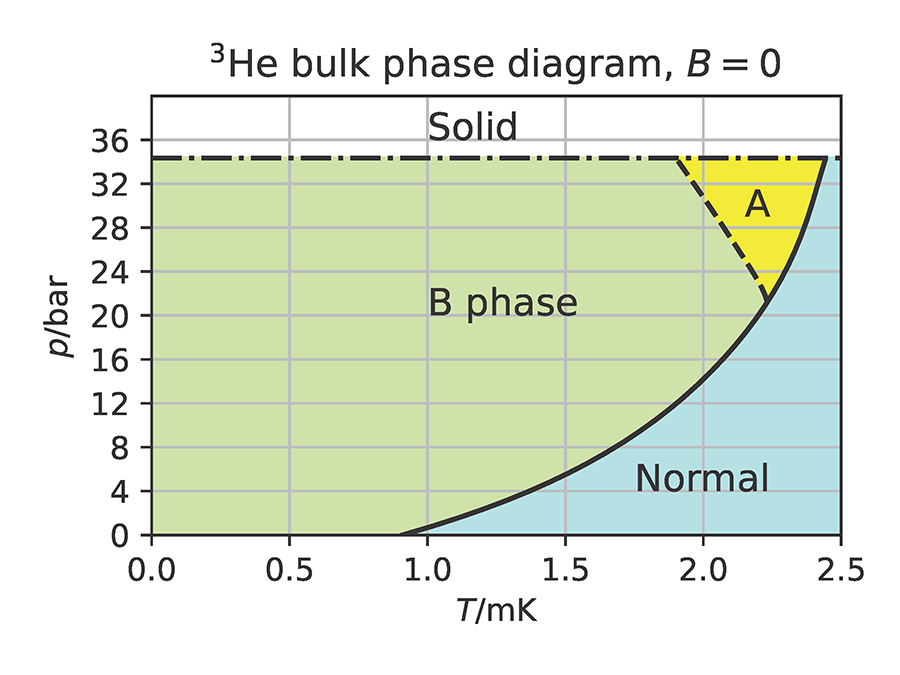
Nucleation theory predicts that the lifetime of the A phase at any temperature or pressure is enormous, far longer than the age of the universe. Yet the transition from A phase to B phase is observed to happen within in a few hours. Thus nucleation theory fails dramatically for superfluid 3He, raising questions as to whether rate calculations for cosmological phase transitions are also incorrect. We might be predicting completely the wrong frequency, or even worse for the programme of searching for new physics, there may be no gravitational waves at all. Hence there is an urgent need to resolve the A-B nucleation puzzle.
Proposed explanations for the puzzle are many and varied. Experiments have potential nucleation seeds in the form of rough surfaces, which are unavoidable in the heat exchanger through which the superfluid is cooled. In experiments designed to isolate the heat exchanger, the leading explanations contend that nucleation is caused by energy deposited by cosmic-ray muons or an energetic particle from radioactive decay in the apparatus. The energy deposited causes the superfluid to heat into the normal phase in a small region, which finds its way to the B phase as it cools. These proposals were named the “Baked Alaska” [citation] scenario and the “Cosmological” [citation] scenario, differing in their description of the physical processes by which the region cools after the energy is deposited. During the 1990s, experiments with radioactive sources were conducted both in stationary and rotating cryostats without reaching a definite conclusion about which scenario is correct.
New techniques, both experimental and theoretical, have become available since the experiments performed in the 1990s. Key to any experiment is the control or elimination of boundary effects. The QUEST-DMC experimental programme does this in two ways. The first, at Royal Holloway, University of London, nanofabricates cells out of a single silicon crystal to make atomically smooth walls and takes advantage of confinement to keep the system in the A phase near the heat exchanger. The other, at the University of Lancaster, uses magnetic fields to isolate metastable A phase from experimental boundaries. HIP’s contribution, working closely with the University of Sussex, is on the simulation side, benefiting from many years of experience in cosmological simulation and Finland’s excellent computational resources at CSC IT Center for Science.
Simulating the A-B transition
Simulation of the dynamics of the A-B transition is technically challenging because the state at each point of the superfluid is described by an 18-dimensional field, or order parameter, describing the spin and the angular momentum of pairs of 3He atoms. One wants to simulate as large a 3 dimensionL region of the experiment as possible. Such simulations require high-performance computational (HPC) resources, both in terms of parallel floating point performance and effective input and output (I/O) for visualization and analysis of the results. To utilize HPC systems with distributed memory, we use the sophisticated lattice field theory library HILA [HILA repository]. The dynamical theory of superfluid 3He, time-dependent Ginzburg-Landau effective theory, then is discretized on the uniform grid offered by HILA and can be run on the largest supercomputers in the world, such as CSC’s Lumi.
As a first test of the approach, we set up a highly disturbed initial state modelling the aftermath of a particle passing through an experimental cell filled with superfluid in the metastable A phase. Out of the random fluctuations of the order parameter we found that bubbles of the B-phase were born (Figure 2). Intriguingly, this points to a different mechanism from either of the two scenarios mentioned above, as the system seems not to enter the normal phase. These preliminary results have been posted to the arXiv repository and submitted to the Journal of Low Temperature Physics.
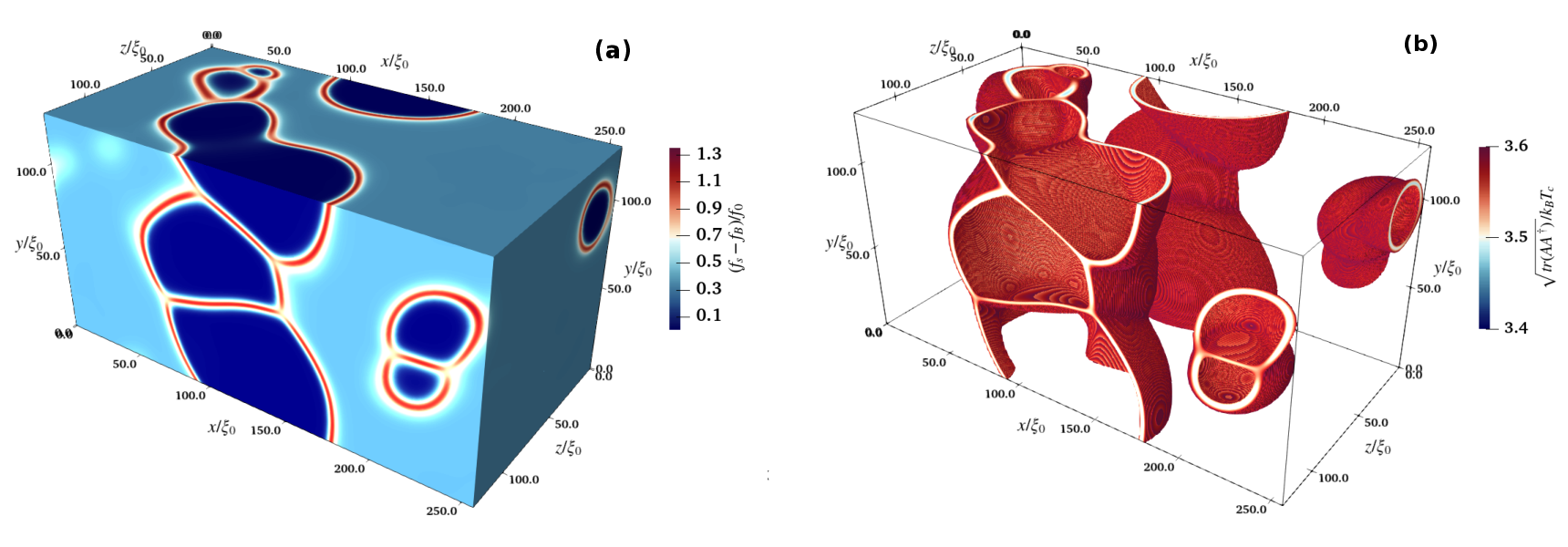
Next steps
The collaboration is holding a meeting at the physics department at the University of Helsinki at the end of this month to discuss the latest results and the next steps. We are planning and conducting more detailed simulations of existing nucleation scenarios, including the “Baked Alaska” model and the “Cosmological” scenario, as well as the different type of nucleation caused by exotic order parameter configurations near boundaries. The experiment at Royal Holloway is in full swing, with dozens of phase transitions observed at different temperatures and, for the first time, different pressures. At Lancaster, the powerful coil magnet is being wound, and the experiment at high magnetic field is expected to start later this year. With this combination of simulation and experiment the A-B nucleation puzzle may finally be solved, and the question mark hanging over LISA’s search for gravitational waves from the early universe removed.
Mark Hindmarsh
Professor, Division of Particle Physics and Astrophysics, University of Helsinki
University of Sussex
HIP Project (Theoretical cosmology)
Kuang Zhang
Research Fellow in Theoretical Physics, Department of Physics and Astronomy, University of Sussex
University of Helsinki
HIP Project